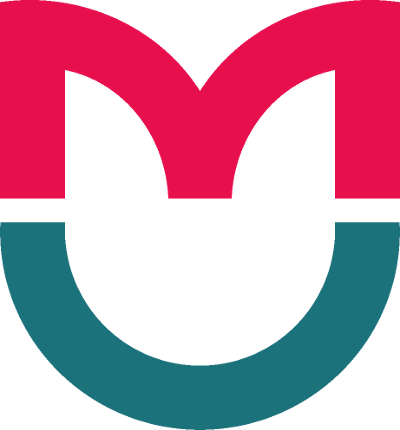
REVIEW
Targeted nanomedicines for applications in preclinical cancer models
1 Department of Oncology, University of Turin, Candiolo, Italy
2 Candiolo Cancer Institute-FPO-IRCCS, Candiolo, Italy
Correspondence should be addressed: Serena Marchió
Institute for Cancer Research and Treatment, University of Torino, 142 Km 3.95, Candiolo, Italy, 10060; ti.ccri@oihcram.aneres
Early diagnosis and effective treatment of cancer are essential to minimize morbidity and mortality. These goals can be achieved by combining 1) disease-specific molecules that may serve as both diagnostic markers and therapeutic targets with 2) imaging and drug delivery tools capable of providing high-performance intervention on diseased sites without (or only marginally) affecting nearby or distant healthy tissues. Despite this broadly accepted assumption, progress in patient-tailored approaches has been relatively slow, particularly due to the paucity of suitable molecular markers. For example, of the ~1,500 proteins proposed as new cancer biomarkers in the decade 2000-2010, only < 20 have been approved by the Food and Drug Administration (FDA) to be used in routine testing [1]. On the other hand, applications of nanotechnology to medicine (collectively defined as nanomedicine) are experiencing a tremendous impact on next-generation cancer management, as demonstrated by the number of ongoing clinical trials and advanced preclinical studies [2, 3]. A nanomedicine is a therapeutic, diagnostic or combined (theranostic = therapeutic + diagnostic) agent embedded in, or otherwise associated to, a nanoparticle to provide better biodistribution, improve the efficacy and/or reduce the toxicity of the agent itself. In our studies, we identified new biomarkers and explored preclinical applications of targeted nanomedicines for cancer imaging (targeted fluorescent nanoparticles) and treatment (targeted drug-loaded liposomes). In this minireview, we outline the principal findings of these studies.
Targeted molecular imaging of metastatic colorectal cancer
Our research group has described a previously unknown complex of α6 integrin and E-cadherin, which is present on the surface of colon cancer cells but not of normal colon cells [4]. We have also identified a specific ligand to this receptor complex, namely angiopoietin-like 6, a factor secreted in high amounts by normal liver and physiologically involved in lipid metabolism. We have demonstrated that this receptor/ligand circuit is operative in secondary tumor spreading: colon cancer cells expose the receptor, normal liver cells secrete the ligand, and their mutual recognition allows cancer cells to colonize the liver and eventually produce a metastatic mass in this site [4].
In addition, we have characterized two angiopoietin-like-6 mimicking peptides that bind the α6 integrin/E-cadherin complex. These peptides, of sequence CGIYRLRS and CGVYSLRS (single letter amino acid code), besides competing with angiopoietin-like 6 for binding the receptor complex (and therefore inhibiting hepatic metastasis), represent potential tools to flag tumor cells that expose both α6 integrin and E-cadherin [4]. So, we have exploited their binding properties to design nanomedicines for cancer imaging [5]. This study was based on silica nanoparticles, which exhibit favorable toxicological profile and biocompatibility in vivo coupled to ease of manipulation in vitro [6, 7]. We produced modular nanosystems to obtain an imaging platform consisting of fluorescent silica-polyethylene glycol (PEG) nanoparticles (SPNs) that expose either the CGIYRLRS or CGVYSLRS peptide on their surface. These SPNs have a silica nucleus associated to one or more alkoxysilane-derivatized fluorescent dyes, included in a micelle of the copolymer Pluronic®F127. In other words, they consist of a PEG shell incorporating a dye-doped silica core. The external PEG provides a standard of stealth polymer for stable dispersion in physiological conditions and for prevention of uptake by the phagocyte system. Moreover, the PEG tails can be derivatized to allow covalent attachment of targeting peptides. Our SPNs have a core diameter of 11 ± 3 nm, a hydrodynamic diameter of 23 nm and are doped with either Rhodamine A (Rhod), Cyanine 5 (Cy5) (single-color), or both (dual-color). Their specificity was first investigated ex-vivo on patient-derived specimens of hepatic metastasis, compared to normal liver and to primary colon cancer (fig. 1). Sections of frozen human tissues were incubated with control (untargeted) and dual-color peptide-targeted (Rhod+Cy5)-SPNs. Nanoparticle selectivity was evaluated by confocal microscopy (imaging; fig. 1 A–D, quantification; fig. 1 E), revealing specific binding of CGIYRLRS- and CGVYSLRS-(Rhod+Cy5)-SPNs on hepatic metastasis (fig. 1 B) compared to normal liver (fig. 1 A) and colon (fig. 1 C), and to the primary tumor (fig. 1 D).
The SPNs were also tested in vivo in a mouse model of pseudo-metastatic tumor (human colon cancer cells implanted into the spleen of non-obese diabetic/severe combined immunodeficient mice, NOD/SCID; fig. 2). Tumor-bearing mice were injected with control and targeted SPNs and signal was detected after increasing circulation times starting at 1 hour. At 6 hours, we observed a substantial reduction in background fluorescence and this signal-to-background ratio remained consistent at 16 and 24 hours. This suggests that clearance of untargeted nanoparticles is accompanied by accumulation of targeted SPNs in metastatic foci, providing a large timeframe for applications to be translated to the clinics. Fluorescence imaging by stereomicroscopy and confocal microscopy confirmed a metastasis-specific accumulation of (Rhod)- CGIYRLRS-SNPs (fig. 2 A, D, G, H), (Cy5)-CGIYRLRS-SNPs (fig. 2 B, E, I, J) and (Rhod-Cy5)-CGIYRLRS-SNPs (fig. 2 C, F, K, L, M, N). A tridimensional reconstruction of several confocal microphotographs showed that the targeted SNPs localize in close proximity of tumor blood vessels (fig. 2 O, P).
The intra-operative use of fluorescence tracers is starting to emerge in prostate, gastric, urinary and ovarian cancers [8–11]. Fluorescent imaging of externally accessible human cancers, namely nonmelanoma skin tumors can be achieved [12] and endoscopic fluorescence imaging systems have also been developed for applications in colon cancer [13]. All these systems are based on untargeted fluorescent tracers, while our SPNs have the additional feature of being molecularly targeted, providing further proof of feasibility for translational consideration.
Targeted drug delivery in metastatic neuroblastoma
In the past years, we and our collaborators have also identified peptides with unique targeting features for applications in tumor treatment. Among these, CPRECES [14] and CNGRC [15] (single letter amino acid code) bind with high selectivity to the tumor endothelial/perivascular markers aminopeptidase A (APA) and N (APN), respectively, so they are optimal for in vivo applications of drug delivery via the circulation.
These two peptides were exploited in a first study aimed to define the preclinical feasibility of targeted nanomedicines for doxorubicin (DXR) delivery to models of infantile neuroblastoma [16]. For this purpose, a synthetic version of each peptide was produced as fusion with human tumor necrosis factor (TNF) and the short linker KY (single letter amino acid code), and then coupled to the PEG tails of stealth liposomes (SL) composed of distearoyl phosphatidylethanolamine (DSPE)-PEG, hydrogenated soy phosphatidylcholine (HSPC) and cholesterol. These SLs were loaded with DXR to obtain the targeted nanosystems CPRECES-SL[DXR] and CNGRC-SL[DXR], respectively, with a size of 90–115 nm, drug entrapment of 95% and peptide coupling of 4 μg/μmol of SL. For pharmacokinetic studies, these SLs were dual-labeled by incorporation of 3H and 14C in cholesterol and DXR, respectively, demonstrating suitable stability and long circulation times (up to 24 hours) [16]. The efficacy of such formulations, either as a single agent or in combination regimens (COMBO), was evaluated in orthotopic models derived by implant of human neuroblastoma cells into the left adrenal gland of nude mice. Starting 21 days after tumor cell implant, mice were treated once a week for 5 weeks with 5 mg/kg DXR (free or liposome-incapsulated), showing that administration of CPRECES-SL[DXR], CNGRC-SL[DXR] and COMBO provided a consistent lifespan extension vs. the free drug (up to 17, 37 and 66 days, respectively; fig. 3) [16].
This work demonstrates that targeting a drug to the tumor microenvironment increases its efficacy and can therefore be exploited for the development of innovative medicines.
Based on these encouraging results, in a successive study we performed combined in vitro/ex-vivo screenings of phage-displayed peptide libraries to identify novel peptide motifs with high specificity for human neuroblastoma [16]. These experiments were designed to isolate peptides capable of binding the whole primary tumor (n = 26 motifs retrieved) or metastatic mass (n = 15 motifs), the primary tumor microenvironment (ME) (n = 57 motifs) or the metastasis ME (n = 23 motifs). The specificity of 5 peptides targeting the metastatic mass (phage clone #14, peptide sequence KSFFLSH), the primary tumor ME (#1, YEGLISR) and the metastasis ME (#5, HSYWLRS; #8, WSWPREL; #10, ALAAHKL) was confirmed ex vivo by binding assays on sections of human stage IV neuroblastoma and in vivo in mouse models. These peptides were therefore synthetized with the addition of an N-terminal (YSHS, single letter amino acid code) and a C-terminal (GGG, single letter amino acid code) linkers and coupled to SLs as described above [16]. The potential efficacy of these nanosystems was tested in an orthotopic model derived from implant of luciferase-transduced human neuroblastoma cells; in addition, a pseudo-metastatic model was obtained by tumor cell injection in the tail vein of nude mice. Orthotopically-implanted mice were treated once a week for 3 weeks starting 21 days after tumor cell implant, and intravenously-implanted mice were treated with the same schedule but starting 4 hours after tumor cell implant. In a first series of experiments, growth of luciferase-expressing orthotopic tumors was monitored by bioluminescence imaging (BLI) at days 26, 33, 40 after implant (5 days after each treatment) showing that 5-SL[DXR] and 10-SL[DXR] were the most efficient in delaying tumor progression, as also confirmed by a whole-body X-ray scan performed one month after the end of treatments (fig. 4 A). Successively, the capacity of targeted formulations to prolong the lifespan of tumor-bearing mice was evaluated in both the pseudo-metastatic (fig. 4 B) and the orthotopic (fig. 4 C) model. Again, treatment with 5-SL[DXR] or 10-SL[DXR] provided a survival advantage to neuroblastoma-bearing mice when compared to control animals or even to animals treated with DXR, either free or included in untargeted liposomal formulations [16].
These preliminary findings were complemented by a successive in-depth characterization of the HSYWLRS peptide (#5 of the previous study) as a targeting moiety in preclinical applications [17]. Binding and internalization specificity was confirmed on additional cell lines and tissue specimens from animal models and human stage IV neuroblastoma. DXR-loaded, peptide-targeted SLs (HSWYLRS-SL[DXR]) were therefore produced and tested as in vivo drug delivery nanosystems. Vascular permeability was evaluated by administration of Evans Blue in neuroblastoma-bearing mice treated with HSYWLRS-SL[DXR], observing a specific increase in dye extravasation and accumulation in orthotopic tumors, but not in non-tumor tissues (fig. 5 A). Treatment with HSYWLRS-SL[DXR] also increased perfusion of tumor blood vessels, as determined by intravenous injection of fluorescein isothiocyanate (FITC)-lectin and analysis of emitted fluorescence by confocal microscopy (fig. 5 B). These phenomena were accompanied by (1) enhanced tumor accumulation of HSYWLRS-SL[DXR], but not of DRX included into an untargeted liposomal formulation, and (2) prolonged animal survival (fig. 5 C) in the absence of toxicity signs such as weight loss (fig. 5 C, inset). Additionally, the therapeutic efficacy of HSYWLRS-SL[DXR] was compared to that of free DXR by monitoring the in vivo growth of orthotopically-implanted, luciferase-expressing human neuroblastoma cells with BLI, revealing that only the targeted liposomal formulation elicits effective antitumor responses (fig. 6 A–B) and prolongs the lifespan of tumor-bearing mice (fig. 6 C). The effect was also validated by positron emission tomography (PET) coupled with glucose consumption measurement. This analysis revealed that treatment with HSYWLRS-SL[:lit_DXR;] led to a substantial inhibition not only of primary tumor growth but also of secondary tumor spreading (fig. 7 A), which was confirmed by animal autopsy in terms of both metastatic foci number (fig. 7 C) and overall metastasis volume (fig. 7 C).
Together, these data support the development of the neuroblastoma-targeting peptide HSYWLRS as a powerful tool for therapeutic applications.
CONCLUSIONS
Early tumor diagnosis and efficient treatment (namely, a treatment that reaches max antitumor efficacy while sparing normal tissues) remain an open medical need. The advent of nanomedicine is delivering new tools that could revolutionize our approach to cancer monitoring and therapy, provided that we identify biomarkers with suitable properties. In fact, while the development of nanomaterials is rapidly expanding, we still need more selective targets to allow patient-tailored approaches. In this perspective, our recent work contributed a number of targets and targeting moieties that were validated preclinically and could be exploited to develop clinical tools.